Development of new photocatalysts for water splitting
The reaction (H2O -> H2 + 1/2O2) that uses light energy to break down water, as shown in Fig. 1, is the most fundamental reaction in photosynthesis and is of academic interest. In addition, these are reactions in which the Gibbs free energy change are very large reactions and are important in terms of the conversion of light energy to chemical energy. Furthermore, the hydrogen obtained from this reaction is very useful as a clean energy and chemical industrial raw material. Therefore, our laboratory conducts water splitting using heterogeneous photocatalysts with semiconductors, as shown in the figure.
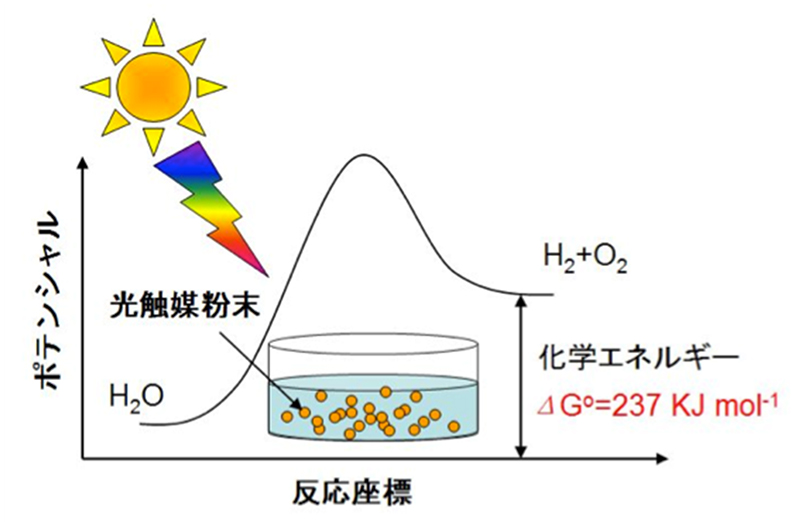
Fig. 2 illustrates the principle of the water decomposition reaction by heterogeneous semiconductor photocatalysts. When a semiconductor is exposed to light with energy above its band gap, electrons in the valence band electrons in the valence band are excited to the conduction band. These excited electrons reduce water to produce hydrogen. Holes formed in the valence band, on the other hand, oxidize water to produce oxygen. In this way, a photocatalytic water decomposition reaction proceeds. This reaction looks simple at first glance. may seem simple at first glance, but in reality, it is extremely difficult because various factors come into play.
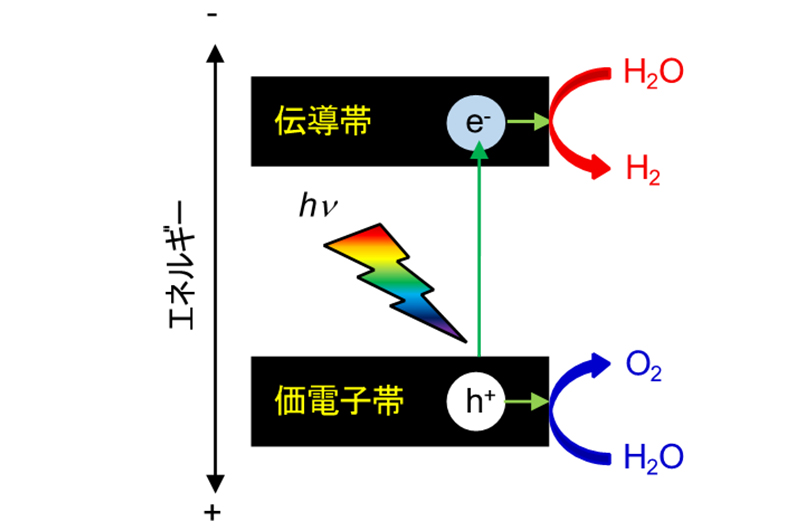
As shown in Fig. 3, sunlight contains a mixture of light with a wide range of wavelengths from infrared to ultraviolet, and it is important to develop a photocatalyst that can absorb many photons. It is important to develop a photocatalyst that can absorb a large number of photons. Therefore, in order to efficiently perform this reaction using sunlight, it is desirable to develop a photocatalyst with high activity that responds to long-wavelength light. From this perspective, the development of photocatalysts to efficiently use sunlight for water splitting has been actively pursued. However, there is still no photocatalyst However, there is still no photocatalyst that can efficiently Therefore, we are working on the development of photocatalysts that are highly active in water photolysis, i.e., artificial photosynthesis, based on our original design guideline. We are conducting research based on our original design guideline. Furthermore, from the viewpoint of synthesis of ceramics under mild conditions, we are establishing a new soft-process synthesis method for photocatalysts. We are also working on the establishment of new soft process synthesis of photocatalysts from the viewpoint of synthesis of ceramics under mild conditions. In addition, from the academic viewpoint of solid surface reaction and photoelectron transfer, we are conducting research as photocatalytic science, incorporating computational science as well. The following is a list of specific research themes. Specific themes are listed below.
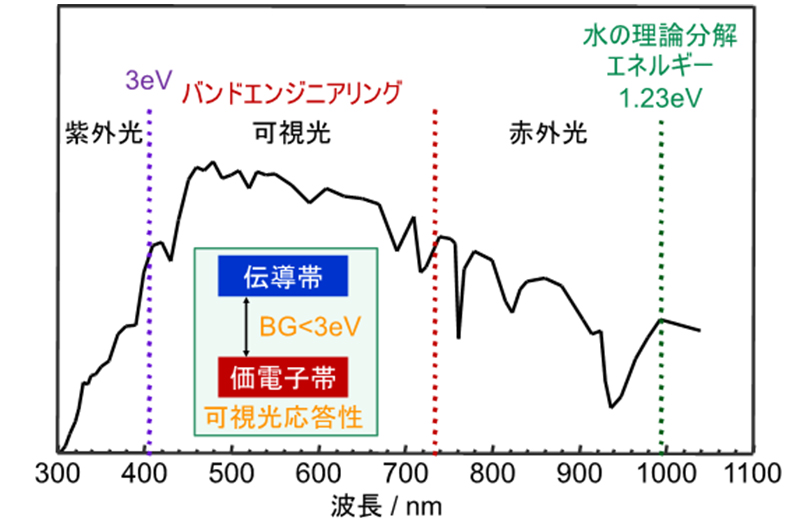
Development of new photocatalytic materials
Our laboratory has constructed a library of photocatalysts as shown in Table 1. Among these, we have found that many of the tantalum-based composite oxides exhibit highly efficient water decomposition activity.
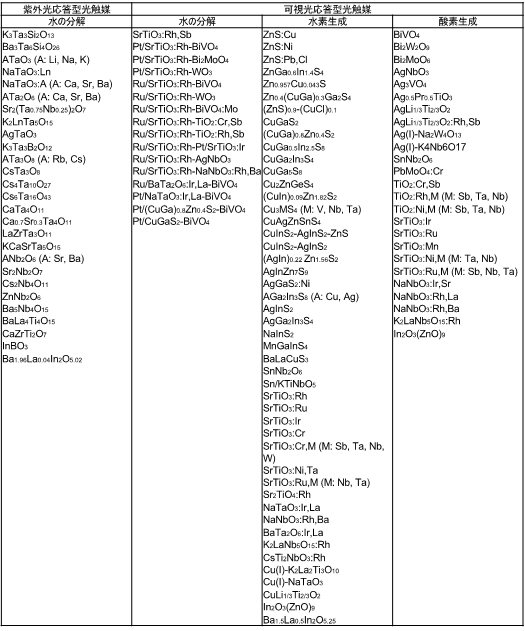
The La-doped NaTaO3 photocatalyst shown in Fig. 4 is highly active, producing hydrogen and oxygen from water at 500 mL-1, 250 mL-1 each (using a 400 W high-pressure mercury vapor lamp). Click here to see a video of the reaction. However, this photocatalyst absorbs only ultraviolet light below 310 nm, so it cannot be expected to be active under sunlight irradiation. However, this photocatalyst absorbs only ultraviolet light below 310 nm.
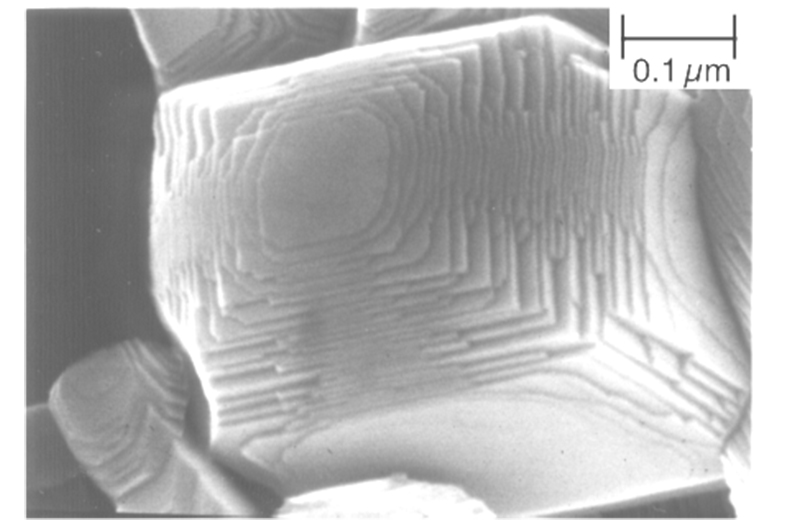
To develop a photocatalyst that can efficiently utilize sunlight, it is important to narrow the band gap of the photocatalyst so that it can use light of longer wavelengths. To achieve this, the following three methods are used. The following three methods can be used to achieve this.
(1) Transition metal doping for photocatalysts with large band gaps
(2)Valence band control
(3)Solid solution formation
(1) Transition metal doping for photocatalysts with large band gaps
Our laboratory has been developing photocatalysts that can utilize long wavelength light by doping metal ions into photocatalysts with a large band gap to form new levels in the band gap. In particular, Rh-doped SrTiO3 photocatalyst, shown in Fig. 5, responds to visible light and shows high hydrogen activity in the presence of reducing agents.
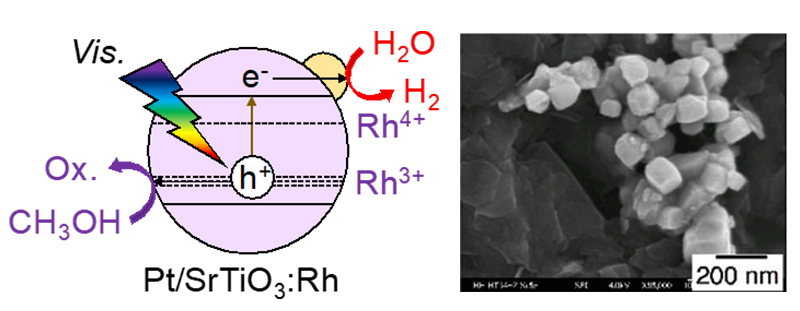
We also found that Rh- and Sb-doped SrTiO3 metal oxide photocatalysts loaded with IrOx auxocatalysts exhibit visible light water splitting activity, as shown in Fig. 6. We also found that Ir-doped SrTiO3 photocatalysts with an Ir co-catalyst exhibit hydrogen activity in the presence of a reducing agent in response to light up to 800 nm.
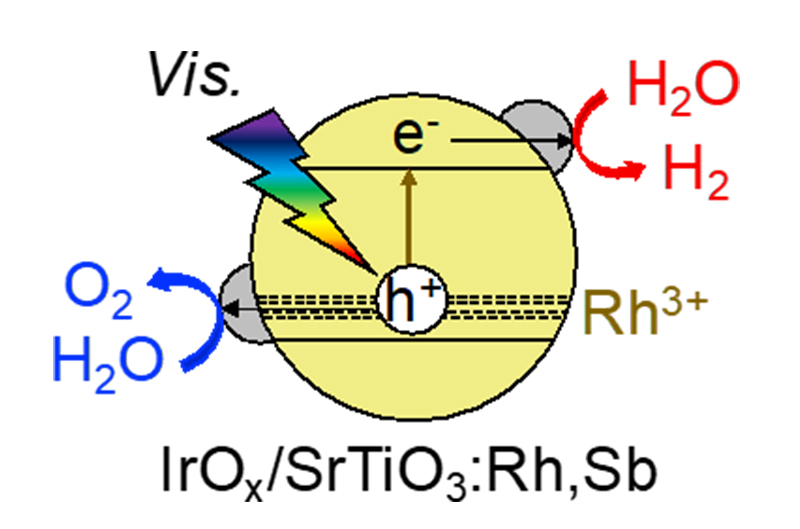
(2) Valence band control
Valence band control is a method of narrowing the band gap of a photocatalyst by forming a valence band with other elements in place of the valence band of the photocatalyst with a wide band gap. In our laboratory, we have developed BiVO4, AgVO3, and SnNb2O6. In particular, BiVO4 shown in Fig. 7 efficiently produces oxygen in response to visible light in the presence of an oxidant.
We also found that AgTaO3 photocatalyst loaded with Rh0.5Cr1.5O3 auxocatalyst exhibited high water splitting activity, albeit under UV light irradiation. Click here to see a video of the reaction.
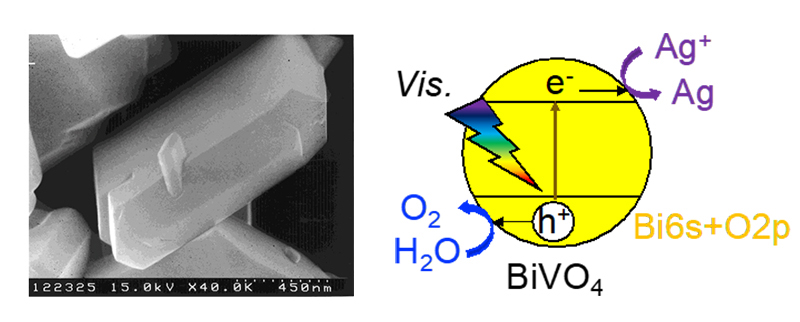
(3) Solid solution formation
Formation of solid solution can also narrow the band gap. In our laboratory, we have developed a photocatalyst combining CuInS2, AgInS2, and ZnS that can efficiently produce hydrogen in the presence of a reducing agent when irradiated with visible light (Fig. 8). In particular, a combination of black photocatalysts, CuInS2, AgInS2, and ZnS, can generate hydrogen in response to light up to 830 nm. Click here to see a video of the reaction.
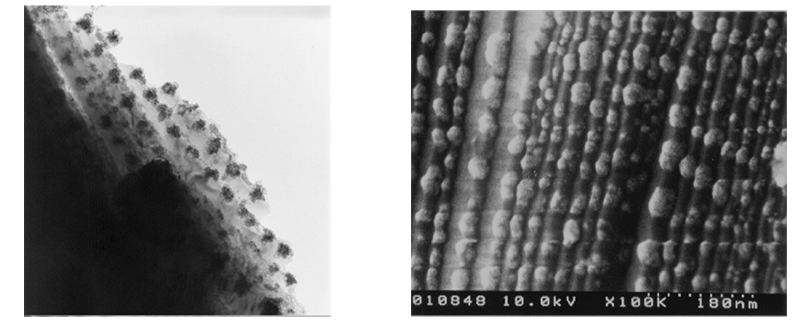
Development of new Z-Scheme system
Our laboratory also studies Z-scheme photocatalytic systems that combine hydrogen-producing and oxygen-producing photocatalysts to mimic the two-step photoexcitation (Z-scheme) photosynthetic mechanism in plants. Fig. 9 shows the reaction mechanism of its Z-scheme photocatalytic system. On the oxygen-generating photocatalyst, the oxidized form of the electron-transfer system is reduced to the reduced form by excited electrons, and water is oxidized by holes to produce oxygen. On the other hand, on a hydrogen-producing photocatalyst, holes oxidize the reductant and excited electrons reduce water on the catalyst to produce hydrogen. The electron transfer system then repeats redox without being consumed, and overall, water splitting proceeds. We have also confirmed the progression of the water splitting reaction under solar irradiation. More recently, we have also studied Z-scheme systems without electron transfer agents.
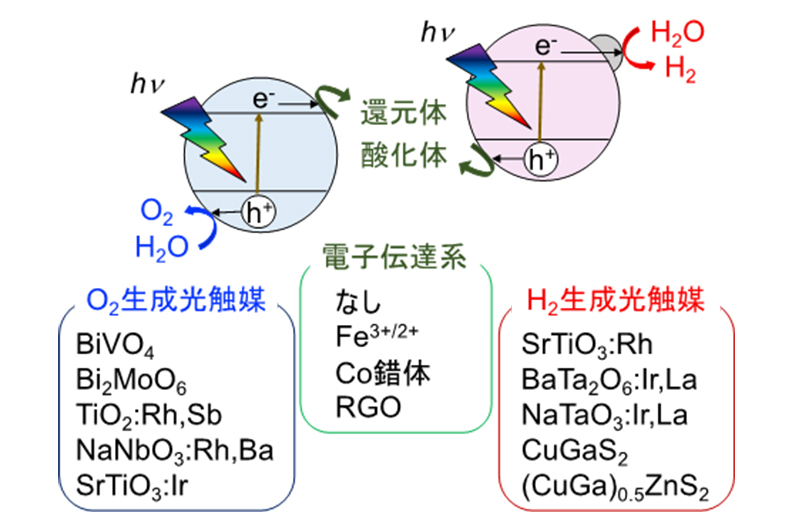
References
- R. Konta, T. Ishii, H. Kato, A. Kudo, J. Phys. Chem. B 2004, 108, 8992.
- R. Asai, H. Nemoto, Q. Jia, K. Saito, A. Iwase, A. Kudo, Chem. Commun. 2014, 50, 2543.
- S. Suzuki, A. Iwase, A. Kudo, Chem. Commun. 2018, 54, 10606.
- A. Kudo, K. Omori, H. Kato, J. Am. Chem. Soc. 1999, 121, 11459.
- R. Konta, H. Kato, H. Kobayashi, A. Kudo, Phys. Chem. Chem. Phys. 2003, 5, 3061.
- Y. Hosogi, H. Kato, and A. Kudo, J. Mater. Chem. 2008, 18, 647.
- Y. Hosogi, K. Tanabe, H. Kato, H. Kobayashi, A. Kudo, Chem. Lett. 2004, 33, 28.
- K. Watanabe, A. Iwase, A. Kudo, Chem. Sci. 2020, 11, 2330.
- I. Tsuji, H. Kato, A. Kudo, Angew. Chem. Int. Ed. 2005, 44, 3565.
- H. Kato, M. Hori, R. Konta, Y. Shimodaira, A. Kudo, Chem. Lett. 2004, 33, 1348.
- Y. Sasaki, H. Nemoto, K. Saito, A. Kudo, J. Phys. Chem. C 2009, 113, 17536.
- Y. Sasaki, H. Kato, A. Kudo, J. Am. Chem. Soc. 2013, 135, 5441.
- A. Kudo, S. Yoshino, T. Tsuchiya, Y. Udagawa, Y. Takahashi, M. Yamaguchi, I. Ogasawara, H. Matsumoto, A. Iwase, Faraday Discuss. 2019, 215, 313.
- H. Kato, M. Hori, R. Konta, Y. Shimodaira, A. Kudo, Chem. Lett. 2004, 33, 1348.
- A. Iwase, A. Kudo, Chem. Commun. 2017, 53, 6156.
- K. Iwashina, A. Iwase, Y. H. Ng, R. Amal, A. Kudo, J. Am. Chem. Soc. 2015, 137, 604.
- T. Kato, Y. Hakari, S. Ikeda, Q. Jia, A. Iwase, A. Kudo, J. Phys. Chem. Lett. 2015, 6, 1042.